The IllustrisTNG project is a suite of state-of-the-art cosmological galaxy formation simulations. Each simulation in IllustrisTNG evolves a large swath of a mock Universe from soon after the Big-Bang until the present day while taking into account a wide range of physical processes that drive galaxy formation. The simulations can be used to study a broad range of topics surrounding how the Universe — and the galaxies within it — evolved over time.
Motivation and Big Ideas
The standard model of cosmology posits that the mass-energy density of the Universe is dominated by unknown forms of dark matter and dark energy. Testing this extraordinary scenario requires precise predictions for the formation of structure in the visible matter, which is directly observable as stars, diffuse gas, and accreting black holes. These components of the visible matter are organized in a 'Cosmic Web' of sheets, filaments, and voids, inside which the basic units of cosmic structure - galaxies - are embedded. To test our current ideas on the formation and evolution of galaxies, we strive to create simulated galaxies as detailed and realistic as possible, and compare them to galaxies observed in the real universe. By probing our successes and failures, we can further enhance our understanding of the process of galaxy formation, and thereby perhaps realize something fundamental about the world in which we live.
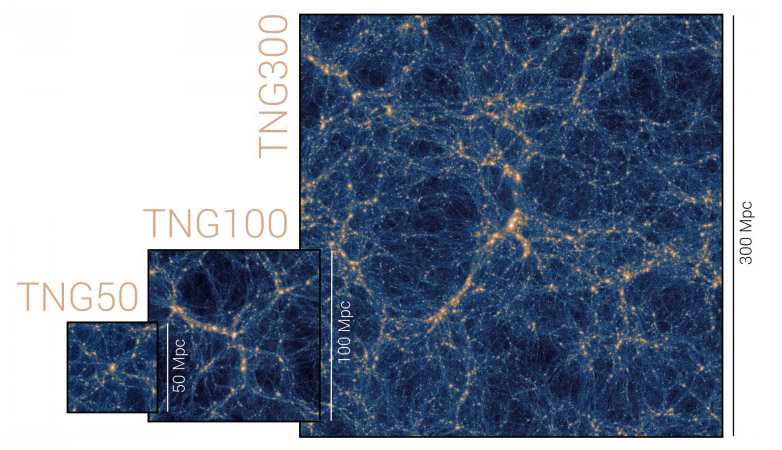
IllustrisTNG Project Overview
The original IllustrisTNG project consists of three volumes, and 18 simulations in total. The individual simulations vary in their physical size, mass resolution, and complexity of physics included. Three physical simulation box sizes are employed: cubic volumes of roughly 50, 100, and 300 Mpc side length, which we refer to as TNG50, TNG100, and TNG300, respectively. The three boxes compliment each other by promoting a focus on various aspects of galaxy formation. The large physical volume associated with the largest simulation box (TNG300) enables the study of galaxy clustering, the analysis of rare objects such as galaxy clusters and provides the largest galaxy sample. In contrast, while the smaller physical volume simulation of TNG50 simulation has a comparatively limited sampling of rare objects, the mass resolution achieved in the smaller volume simulations is a few hundred times higher than the larger volume TNG300 simulation. The TNG50 volume therefore enables a more detailed look at, e.g., the structural properties of galaxies, the detailed structure of gas around galaxies, and the convergence of our physical model. The central volume simulation, TNG100, falls between these two limits. Importantly, the TNG100 volume uses the same initial conditions (adjusted for updated cosmology) as used in the original Illustris simulation, which facilities clean comparisons between the original Illustris results and the updated TNG model.
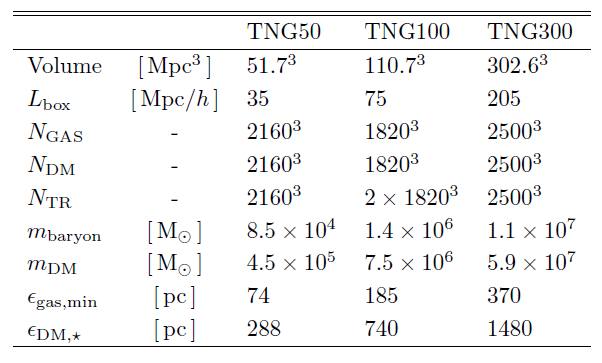
Each of the three simulation boxes has been run at three resolution levels. The highest resolution simulations employ more than 20, 10, and 30 billion resolution elements for the TNG50, TNG100, and TNG300 boxes, respectively. This leads to baryon and dark matter mass resolutions as shown in the table above. Sampling across different resolution levels within the same physical simulations enables clear analysis of the resolution dependence of our results. We employ a physical model that is deliberately constructed to not require parameter tuning as the simulation resolution is varied. So, while the mass resolution between the highest TNG50 run and lowest TNG300 run is separated by a factor of more than 10,000, the parameters employed in the model go unchanged. Comparing the results at different resolution levels helps to evaluate the performance and assess the predictive power of our model. While the details of certain results, such as galaxy stellar masses, change with resolution level, most of the results change in predictable and understandable ways that allow us both understand and correct for the finite resolution of our simulations.
Finally, all of the simulations have "dark matter only" counterparts to their "baryonic physics" runs just described. Dark matter only simulations give predictions for how the large scale structure, the clustering of galaxies, the shapes of halos, and so forth would evolve in a Universe constructed only of dark matter. These predictions are useful in part because they are relatively clean, owing to their sole dependence on the gravitational assembly of dark matter halos. However, at the same time, such models fully neglect the important, but uncertain, impact of baryons on the growth of galaxies. Having side-by-side dark matter only and full physics simulations allows us to directly compare and understand the impact that baryon physics has on a broad range of our results.
The TNG50 Simulation
TNG50 is a new class of cosmological volume simulation -- it has been designed to overcome the traditional limitation of compromising volume versus resolution, by simulating a large, fully representative cosmological volume at a resolution which approaches or even exceeds that of modern "zoom" simulations of individual massive galaxies. The simulation realizes a 50 Mpc box sampled by $2160^3$ gas cells, with a corresponding baryon mass of $8 \times 10^4 M_\odot$ (see table above). The median spatial resolution of the star-forming ISM gas is ~100-140 parsecs across cosmic time. It enables us to obtain unparalleled detail, providing a view into the structure, chemo-dynamical evolution, and small-scale properties of galaxies -- the image below shows two massive disk galaxies from the simulation to highlight its ability to resolve internal structural details such as spiral arms, bulges, and nuclear bars, together with the extremely thin scale-heights of galactic disks.
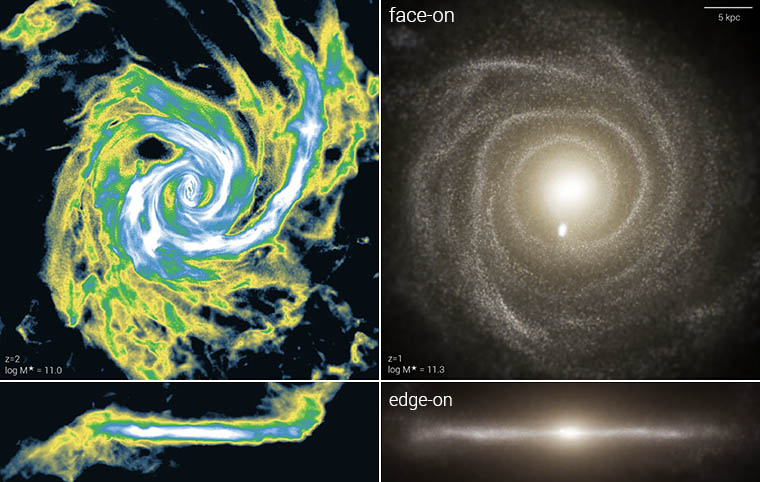
TNG50 contains roughly 100 Milky Way mass-analogs, enabling detailed comparisons to our own galaxy at z = 0. It also hosts one massive galaxy cluster with a total mass ~ $10^{14}$ solar masses, a Virgo-like analog, and dozens of group sized halos at ~ $10^{13} M_\odot$. All of these massive objects are simulated at higher numerical resolution than in any previously published study, enabling studies not only of the gaseous halos and central galaxies, but also of the large populations of their satellite galaxies. Its coverage in redshift range and galaxy stellar mass enables us to make quantitative predictions for signatures observable with the James Webb Space Telescope (JWST), as well as recent ground-based IFU instruments such as MUSE, SINFONI, and KCWI. The key science drivers of TNG50 focus not only on the present day (z = 0), but also at earlier epochs, from cosmic noon (z ~ 2) through reionization (z ~ 6).
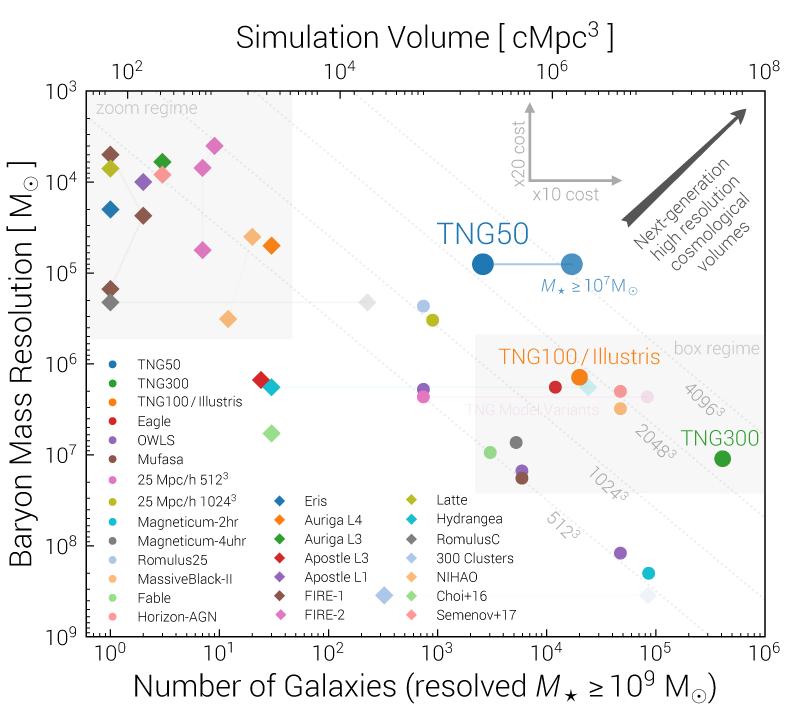
The TNG50 simulation occupies a unique combination of large volume and high resolution, the figure above places it into context. We show TNG50 (dark blue circle) in comparison to other cosmological volumes (circles) and zoom simulation projects (diamonds) at z ~ 0. The x-axis shows an effective volume, given in terms of the total number of resolved galaxies with stellar mass greater than $10^9 M_\odot$. The y-axis shows the resolution, given in terms of the mass of the baryonic mass element. Moving towards the upper right corner represents the frontier for next-generation high-resolution cosmological volume simulations, an extraordinarily intensive computational undertaking which must take advantage of some of the largest supercomputers available.
TNG50 is the third and final simulation of the original IllustrisTNG project.
TNG Spin-off Projects
Since the completion of the original IllustrisTNG simulation suite, a number of further, spin-off projects have been undertaken using the TNG galaxy formation model. These simulations apply the TNG model to regimes, or applications, not accessible by the original TNG50+100+300 volumes.
- CAMELS (2020) - a suite of many, small volume ($\rm{25 Mpc/h}$) simulations, varying cosmological and feedback related parameters, i.e. perturbations on the fiducial TNG model, among others. Focused on machine learning applications and cosmological inference.
- THESAN (2021) - a study of cosmic reionization, combining the fiducial TNG model with a radiative transfer method down to redshift $z \sim 5$, offering a full radiation-hydrodynamical simulation to study the high-redshift galaxy population and the process of reionization.
- MillenniumTNG (2022) - a realization of the original Millennium volume with the fiducial TNG model ($\rm{MTNG740}$), offering large-volume statistics and cosmological applications such as galaxy clustering, the galaxy-halo connection, and the impact of baryons.
- TNG-Cluster (2023) - a simulation including several hundred massive galaxy clusters, $M_{\rm halo} \sim 10^{15} \rm{M}_\odot$, with the fiducial TNG model, designed to study the physics of the intracluster medium, galaxy evolution in dense environments, and cluster cosmology.
How does TNG relate to the original Illustris simulation?
The IllustrisTNG project is the successor of the Illustris simulation. It uses an updated 'next generation' galaxy formation model which includes both new physics as well as refinements to the original Illustris model. The TNG effort is a simulation campaign which:
- (i) retains the fundamental approach and physical model flavor of Illustris,
- (ii) alleviates many Illustris model deficiencies with respect to benchmark observations, and
- (iii) significantly expands the scope with simulations of larger volumes, at higher resolution, and with new physics.
As in Illustris, we follow the coupled dynamics of DM and gas with the robust, accurate, and efficient quasi-Lagrangian code AREPO. In this approach, an unstructured Voronoi tessellation of the simulation volume allows for dynamic and adaptive spatial discretization, where a set of mesh generating points are moved along with the gas flow. This mesh is used to solve the equations of ideal magnetohydrodynamics (MHD) using a second order, finite volume, directionally un-split Godunov-type scheme. The gravitational force is calculated with a split Tree-PM approach, where long-range forces are calculated from a particle-mesh method, and short-range forces are calculated with a hierarchical octree algorithm. The scheme is quasi-Lagrangian, second order in both space and time, uses individual particle timestepping, and has been designed to efficiently execute large, parallel astrophysical simulations on modern supercomputer architectures.
On top of this numerical framework, the TNG galaxy formation model includes the key physical processes needed to study the formation and evolution of galaxies:
- Microphysical gas radiative mechanisms, including primordial and metal-line cooling and heating with an evolving background radiation field.
- Star formation in the dense interstellar medium.
- Stellar population evolution and chemical enrichment following supernovae Ia, II, and AGB stars, individually tracking elements: H, He, C, N, O, Ne, Mg, Si, and Fe.
- Stellar feedback driven galactic-scale outflows.
- The formation, merging, and accretion of nearby gas by supermassive blackholes.
- Multi-mode blackhole feedback operating in a thermal 'quasar' mode at high accretion states, and a kinetic 'wind' mode at low accretion states.
- The amplification of cosmic magnetic fields from a minute primordial seed field at early times.
Scientific Goals
The goals of constructing such a large and ambitious simulation suite are to shed light on the physical processes that drive galaxy formation, to understand when, why, and how galaxies are evolving into the structures that are observed in the night sky, and to make predictions for current and future observational programs to broaden and deepen our understanding of galaxy formation. These goals are achieved not in a single step, but rather through a series of extended analyses of the simulations, each targeting specific science questions. Some of the first questions that have been specifically addressed using the TNG suite are characterizing the stellar masses, colors, and sizes of galaxies, understanding the physical origin of the heavy element (metallicity) distribution in galaxies and galaxy clusters, drawing connections between the presence of dynamically important magnetic fields and the observed radio emission from galaxies, and the clustering signal of galaxies and matter on large scales. Subsequent studies are expected to canvass an even broader range of topics.
The core power of simulation suites — such as TNG — is that the depth of information on the simulation is much deeper than what is accessible observationally alone. For example, one of the most important features of simulations is their access to the time domain. While observations of galaxies can be carried out at different redshifts to give census data about galaxy populations at different stages of their evolution, the timescale over which galaxies evolve (millions, or even more, years) is simply far too long to follow directly observationally. Instead, in observations, bold assumptions are required to infer how census data at different observational epochs leads to a physical picture of galaxy evolution. In stark contrast, simulated galaxy populations in the TNG suite can be directly tracked in time so that an unambiguous and clear picture of their evolutionary history can be pieced together. This facilitates, e.g., clear predictions for the size evolution of galaxies, which would be difficult to directly obtain observationally. Using the simulation’s natural access to the time domain can help guide observational efforts for sussing out physical evolution trends within their multi-epoch observational data.
In addition to the time domain, the simulations also provide unambiguous predictions for physical quantities that might be difficult to derive observationally. For example, observational gas phase or stellar metallicity measurements are complicated derivative quantities that arise out of spectral energy distribution (SED) fitting, or line emission fitting. While significant effort has been put into refining these measurement procedures to the highest possible level of accuracy, significant systematic uncertainty still surrounds the observational measurement methods. In contrast, the TNG simulations make clear and direct predictions for these quantities.
The TNG simulations can therefore be used in concrete ways to build physical models of galaxy formation as well as to aid in the physical interpretation of observational data. The direct knowledge from the simulation can be used to detail the shape of galaxy stellar profiles, examine the color evolution of galaxies, or even characterize the evolution of the baryon acoustic oscillation signal in galaxy clustering data. The central goal of the TNG project is to create a broad tool that will further our understanding of galaxy formation both through direct analysis of the simulation, as well as through assisted interpretation of observational data.
Early Science Results from TNG100 and TNG300
The IllustrisTNG project addresses a number of open questions ranging from galaxy formation and evolution to galaxy clusters and the large scale structure of our Universe. Its wealth of data will be used to answer a large number of scientific questions in these fields. To exemplify how TNG can deepen our understanding of the Universe we summarize some of the first results of the simulations here.
Galaxy formation
One of the main motivations for the IllustrisTNG project is to deepen our understanding of how galaxy formation works. The simulation follows the evolution of dark matter and gas from the early universe until today, self-consistently modeling gravitational interaction, as well as the (magneto-)hydrodynamical interactions of the gaseous component. On top of this, TNG models radiative cooling of gas and the formation of stars and supermassive black holes as well as their feedback and chemical enrichment effects on the host galaxy. This implies that we follow all the main ingredients relevant for galaxy formation in a single simulation, and are therefore able to assess the importance of all these components and their complex interplay on various observable properties.
One of the key properties of a galaxy is its stellar content and how it is distributed. In particular, the distribution of stars in galaxies of different mass is very different: Milky-Way size galaxies mainly build up their stellar content via gas cooling and in-situ star formation. This leads to a centrally peaked stellar mass profiles with an average power-law slope of -5, which implies that on average more than 90% of the stellar mass of such a halo is located within 10% of the halos’ virial radius. The stellar content of the most massive, galaxy cluster size, halos however, is in large parts (80% of the final stellar mass) brought to them via merging with already existing lower mass galaxies. The different physical behavior of stars compared to diffuse gas leads to a significantly different radial distribution of stars, in particular a more pronounced diffuse stellar component. The average power-law slope of the stellar density profile of such a galaxy cluster is around -3, which implies significantly more stars in the outer regions: only about 50% of the stellar mass of galaxy clusters is located within 10% of the virial radius, while the other half is a diffuse component outside this radius and observationally very difficult to detect. However, modeling this correctly in observations is crucial to infer accurate total stellar masses for these systems. To enable this in future observations, we provide analytical fitting functions inferred from TNG to correct for the undetectable components in Pillepich et al. (2017), together with a detailed quantitative analysis of the stellar content of massive halos.
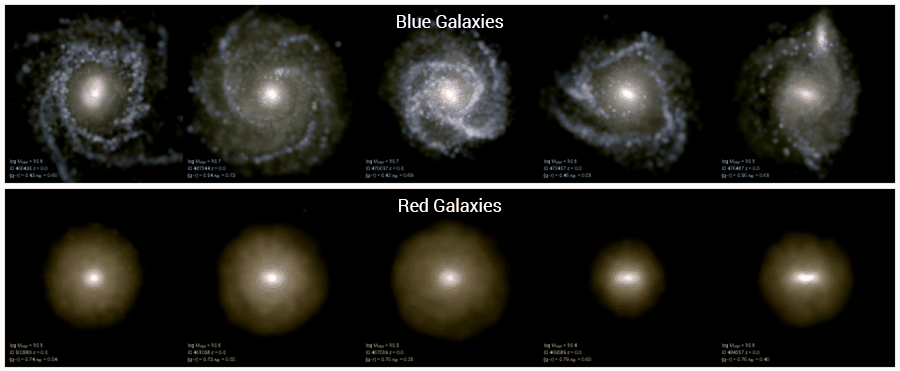
Apart from the total mass of the stars and their distribution, the color at which they collectively shine is one of the most evident observational properties of galaxies. The overall color of a galaxy depends on the collective properties of all stars, in particular on their age and chemical composition. On top of this, the apparent color of a galaxy can change due to the presence of dust, both, close to the stars or in the interstellar medium. In Nelson et al. (2017), we produce mock observations of our simulated TNG galaxies, taking all these effects into account, and compare the resulting color distribution with distributions of observed galaxies from the Sloan Digital Sky Survey (SDSS). The overall agreement of simulated and observed samples is unprecedented. In particular, the g-r color distribution functions in different stellar mass bins are in excellent agreement with observations, with small second-order discrepancy in the slope of the red galaxy population in the g-r vs stellar mass plane, possibly due to aperture effects. This indicates that the underlying physical quantities, i.e. the mean stellar age and stellar metallicity, are also well-reproduced in the simulations, though care has to be taken when comparing these properties directly to observationally inferred ages and metallicities.
The main reason why some galaxies are red is that their stellar populations are very old and all massive stars, which would contribute to the blue light, have already ceased. This leaves only moderately massive and low-mass stars behind, which cause a red appearance. From a galaxy formation point of view, this can only be achieved if there is no formation of new stars in the galaxy. The gas residing in these galaxies however, has a natural tendency to cool and collapse via gravitational instabilities, which ultimately will lead to the formation of new stars. The key ingredient in the simulation to prevent this from happening are feedback effects from supermassive black holes, which become very efficient at a specific mass scale and drastically reduce star-formation in the massive galaxies. In TNG, the transition to a low accretion state and an associated, highly efficient kinetic wind feedback is key for a sharp transition and the buildup of a bimodal galaxy color distribution. The transition time of galaxies evolving from blue, star-forming to red, quiescent galaxies varies significantly from galaxy to galaxy, with a median of 1.6 Gyr. The high scatter in transition time originates from the diverse paths that individual galaxies take through the color-stellar mass plane, which, alongside with a detailed analysis of the low-redshift galaxy color distribution, is presented in Nelson et al. (2017).
Another very important property of stars inside galaxies are the abundances of individual chemical elements, which give important insights into how heavy elements in the Universe form. In TNG, we follow three main enrichment channels that return heavy elements to the interstellar medium: Enrichment from core-collapse supernovae (SNcc), enrichment from type Ia supernovae (SNIa) and enrichment from stellar winds from asymptotic giant branch (AGB) stars. Each of these enrichment channels releases a different chemical composition of elements to the surrounding interstellar medium, which, for SNcc and AGB star enrichment also depends on the properties of the stellar population they originate from. In Naiman et al. (2017), we study the abundance of magnesium (Mg) and iron (Fe) as a proxy of the relative enrichment contribution of SNcc to SNIa as a function of the Fe over hydrogen (H) fraction, a proxy for the overall enrichment. TNG broadly recovers the observed trend, however with an offset normalization, which indicates that the SNIa rate used in TNG might be too low by a factor of a few.
Looking at very rare elements, such as Europium (Eu), which is assumed to be created in neutron star — neutron star mergers, we are able to obtain important insight about the mixing of elements in the interstellar medium during phases of intense star formation. The most Eu enriched gas (high Eu/Fe abundances) originates from starbursts around redshifts 2-4, at the peak of the cosmic star formation history. Interestingly, however, the Eu/Fe ratio does not show a trend with assembly history or present-day galactic properties of the host galaxy in Milky Way-sized halos, indicating that the precise assembly history does not influence the Eu enrichment. The simulated Milky-Way sized galaxies do, however, show a negative trend of the Eu/Fe ratio with star formation rate, suggesting that an increased level of star formation reduces the Eu/Fe enrichment. Possible mechanisms causing this trend, as well as detailed enrichment distributions, are presented in Naiman et al. (2017).
Galaxy Clusters
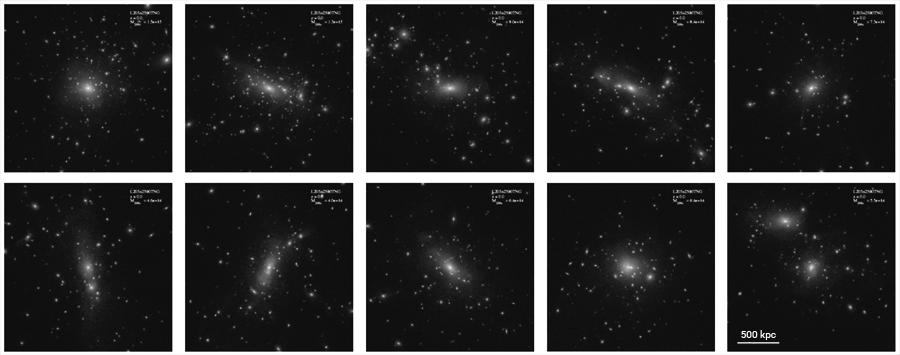
Galaxy Clusters are the largest collapsed objects in our Universe. They do not only contain hundreds of member galaxies (shown above), but also a large reservoir of dilute, hot intra-cluster gas. Observations of this gas component yielded a number of puzzling results which up to now theoretical astrophysicists struggle to explain. One of these results is the existence of extended radio-emission from some galaxy clusters, which is a sign for the presence of magnetic fields and high-energy electrons in these systems. As IllustrisTNG models the presence and amplification of primordial magnetic fields during the collapse of structure in the early universe, we are able to self-consistently study the magnetic properties of the gas in different environments: in low-density regions and cosmic filaments, the magnetic fields closely follow the expectation from adiabatic compression of the primordial field during structure formation, keeping the orientation. In collapsed objects however, where the density is significantly higher, there is an efficient amplification of the magnetic field to about 5 orders of magnitude above the value expected from adiabatic compression alone. The topology of the magnetic field in these regions is consequently strongly correlated with the topology of the gas-flows: the magnetic field in disc galaxies is ordered and disc-like with field strengths of about 10 micro-Gauss, while the magnetic field in elliptical galaxies is unordered, reflecting the chaotic gas motions in these systems.
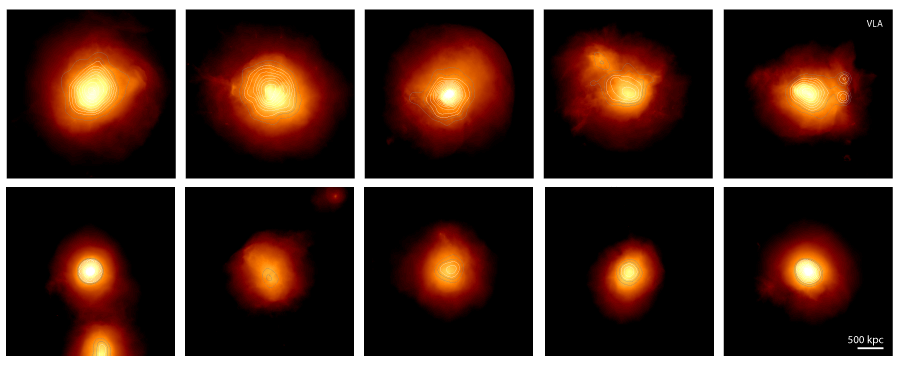
Applying a simple model for relativistic electrons in these systems, it is possible to derive a radio-flux for the simulated galaxy clusters and compare them to observations. In Marinacci et al. (2017) we created these mock radio observations for resolution and sensitivity parameters of VLA, LOFAR, ASKAP and SKA observations. From the simulations it becomes clear that current observations are just able to probe the most radio-luminous objects and the increased sensitivity of future telescopes will open up the possibility to probe the radio emission with far better statistics, which is essential to deepen our understanding of the magnetic fields and relativistic electron population in galaxy clusters. The simulated radio-emission in TNG is broadly consistent with observations, and the clusters obey observed scaling relations between radio and X-ray emission and as well as between radio emission and the Sunyaev-Zel’dovich Compton parameter. However, a more detailed analysis shows some discrepancies, possibly highlighting the need to treat populations of high-energy electrons in these simulations more accurately to provide an adequate theoretical counterpart to future radio telescopes such as SKA. More details on the magnetic field properties and the radio emission in TNG can be found in Marinacci et al. (2017).
Cosmology
Our understanding of the large scale structure, the Cosmic Web and the evolution of the Universe as a whole has made enormous progress in the past decades. The Lambda-Cold-Dark-Matter theory of cosmology is very successful in explaining observations of the primordial chemical abundances, the cosmic microwave background radiation, the expansion history of the universe and the statistical properties of large scale structure. However, there is still a fundamental lack of knowledge, for example on the nature of the so-called dark energy, which drives the accelerated expansion in our Universe. To pin down its physical properties, large observational efforts are taken to further constrain its effects and by this narrow down the number of possible models. Among these efforts, there are large galaxy redshift surveys like EUCLID, DES or eBOSS which will map out the large-scale structure of our universe with unprecedented accuracy. These missions, however measure only the stellar light component of the universe, which, to some extent is not completely equivalent to the overall matter distribution, which is the relevant quantity for cosmological measurements. With TNG, in particular TNG300, hydrodynamical simulations have reached a sufficient volume and resolution to study clustering of all matter components in the Universe on the relevant scales. This means that these kind of simulations have come to a point where they can complement other methods traditionally used in this field, such as for example semi-analytic models, sub-halo abundance matching and halo-occupation distribution approaches. Among these different types of modeling, the TNG simulations provide the most complete and self-consistent approach to following the emergence and evolution of the large-scale structure in our Universe, and can therefore test the assumptions made in the other approaches. Alongside with this, TNG is able to inform about observational biases depending on sample selection and as a function of scales. For example, TNG300 is just large enough to assess the biases at the scales of the baryonic acoustic oscillations (BAO). Its location sensitively depends on cosmological parameters, but only very weakly on galaxy formation physics, making it an ideal probe of cosmology. In Springel et al (2017), we find that the location of the BAO feature can vary up to 6%, depending on tracer used, but can be corrected for by template fitting.
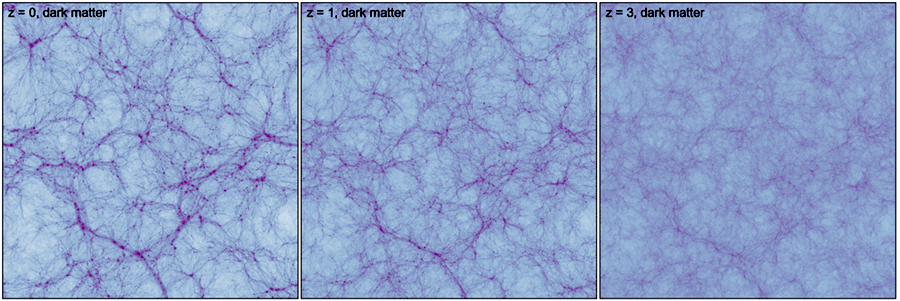
Another effect only hydrodynamical simulations are able to predict self-consistently is the back reaction of the baryonic components on the underlying dark matter distribution, as well as on the overall matter distribution: we find that its impact on the total matter power spectrum becomes >10% at scales with k > 5 h/Mpc at redshift zero, but an effect on the percent level to far larger scales. A detailed analysis on the matter and galaxy clustering is presented in Springel et al. (2017).
Future directions?
The emergence of cosmological hydrodynamical simulations as powerfully predictive theoretical models was embodied in recent projects (from 2013 - 2016) such as Illustris, EAGLE, Horizon-AGN, Magneticum, and MassiveBlack-II. In concert with other large-volume efforts these programs have convincingly demonstrated that hydrodynamical simulations of structure formation at kilo-parsec spatial resolution can reasonably reproduce the fundamental properties and scaling relations of observed galaxies.
In TNG we push these types of simulations to new limits - in size, resolution, and physical fidelity. However, for cosmology, the TNG300 volume is still relatively small. At the other extreme, for studying the properties of the dense and cold phases of the interstellar medium, the resolution and physical model assumptions of TNG50 are still relatively coarse. Therefore, the future steps for such simulations encompass a combined approach of improved and additional physics together with ever more ambitious numerical realizations which continue to take advantage of the increasing computational power of the world's fastest supercomputer systems.